Green Business Talks
The rate of change since the mid-20th century is unprecedented over millennia - NASA
Earth's climate has changed throughout history. Just in the last 800,000 years, there have been eight cycles of ice ages and warmer periods, with the end of the last ice age about 11,700 years ago marking the beginning of the modern climate era — and of human civilization. Most of these climate changes are attributed to very small variations in Earth’s orbit that change the amount of solar energy our planet receives.
Takeaway
-
While Earth’s climate has changed throughout its history, the current warming is happening at a rate not seen in the past 10,000 years.
-
According to the Intergovernmental Panel on Climate Change (IPCC), "Since systematic scientific assessments began in the 1970s, the influence of human activity on the warming of the climate system has evolved from theory to established fact.
-
Scientific information taken from natural sources (such as ice cores, rocks, and tree rings) and from modern equipment (like satellites and instruments) all show the signs of a changing climate.
-
From global temperature rise to melting ice sheets, the evidence of a warming planet abounds.
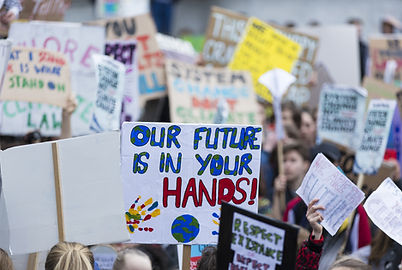
About Us
Search Results
17 results found with an empty search
- Why Green Hydrogen Matters in the Energy Transition?
The world is in a critical fight against climate change, and the transition to clean energy has never been more urgent. Green hydrogen stands out as a transformative force among the many solutions being explored. But what is green hydrogen, and why does it matter so much in the energy transition? Come let's dive into the topic, Hydrogen is not a natural fuel source—it doesn’t exist freely in the earth’s crust. Instead, it must be produced using energy. One of the cleanest ways to produce hydrogen is through electrolysis , where water is split into hydrogen and oxygen using renewable electricity. This process produces what we call " green hydrogen ," a fuel source with no direct carbon emissions. The most common renewable energy that is practically implemented in the market is Electric Vehicles (Source is solar). Researchers compare the efficiency between EVs and Green Hydrogen. Practical Comparision Electric Vehicle: When you charge an EV, some energy is lost during transportation and storage, but the overall efficiency is still around 80%. Charging infrastructure for EVs, such as charging stations, is rapidly expanding and becoming increasingly cost-effective. Green Hydrogen: Renewable electricity is used to produce hydrogen through electrolysis, resulting in energy losses of 30 to 40% . The hydrogen must then be compressed, stored, and transported, which adds to the inefficiencies. Finally, hydrogen is converted back into electricity in a fuel cell to power the vehicle, with an additional energy loss of about 40% . The cumulative losses make hydrogen-powered vehicles less efficient overall compared to EVs. However, they remain critical for sectors where batteries are impractical, such as long-haul trucking, shipping, and aviation. Despite this less efficiency do you know why green hydrogen is important? The answer lies in unique applications. While electricity is ideal for personal vehicles and household energy use, green hydrogen can tackle challenges in hard-to-electrify sectors , such as Steel and Cement Manufacturing: High-temperature industrial processes require energy-dense fuels like hydrogen to replace coal. Long-Distance Transport: In aviation, shipping, and heavy trucking, the weight and size of batteries make hydrogen an attractive alternative. Energy Storage: Hydrogen offers a solution for storing surplus renewable energy for use during periods of low sunlight or wind. Where do we stand now? The journey toward widespread adoption of green hydrogen has only just begun. In 2023 , global production of green hydrogen was less than 100,000 tonnes , with the majority concentrated in China. Most hydrogen today is still produced using fossil fuels, which emit carbon in the process. Looking forward, the world needs to scale up hydrogen production dramatically to meet climate goals. By 2050 , experts project a requirement of 500 million tonnes of green hydrogen annually to support the transition to a low-carbon economy. Overcoming the Roadblocks? The road to green hydrogen dominance is not without challenges: High Production Costs: Electrolysis demands vast amounts of renewable electricity, which currently makes green hydrogen more expensive than hydrogen derived from fossil fuels. Infrastructure Gaps: Transporting and storing hydrogen requires specialized pipelines, pressurized tanks, and other infrastructure that is underdeveloped and costly to implement. Energy Losses: With every energy conversion step, losses accumulate. This reality underscores the importance of minimizing waste in hydrogen production and usage. Why does Green Hydrogen Still Matter? Despite these obstacles, green hydrogen is a cornerstone of the energy transition. It’s not about replacing electricity but complementing it. Green hydrogen’s ability to decarbonize heavy industry, store renewable energy, and power long-haul transport makes it indispensable. Governments, businesses, and researchers around the globe are already investing in scaling up hydrogen technology. From renewable-powered electrolysis plants to innovative hydrogen storage solutions, these efforts are paving the way for a cleaner, more sustainable future. Green hydrogen may not yet be as efficient as direct electrification, but its ability to address the toughest decarbonization challenges—from heavy industry to long-haul transport—makes it indispensable. By investing in green hydrogen today, we are building the foundation for a sustainable and resilient energy future. Together, we can transform ambition into action and secure a greener tomorrow.
- Carbon Capture Utilization and Storage in Offshore Platform
With the development of offshore oil and gas fields, more and more offshore oil and gas fields are found to have high carbon dioxide (CO2), and the carbon dioxide content of some offshore fields is up to 40%. Especially in offshore gas fields, the high content of CO2 has a potential corrosion risk to the upper structures and pipeline. But CO2 cannot be emitted directly into the atmosphere, it is considered to be a major contributor to the greenhouse effect and climate change. Therefore, the correct separation and emission of CO2 have become the key problem to be solved by offshore oil and gas fields. The latest assessment report of the Intergovernmental Panel on Climate Change (IPCC) also shows that to achieve the global temperature limit of 1.5 ℃, global CO2 emissions will need to be reduced by about 45% in 2030 compared to 2010, and “net zero” emissions will need to be achieved in 2050. The CO2 produced by offshore gas fields can be buried on land or subsea . From the engineering and economic point, it is ideal to inject CO2 on land or near shore. Generally, before the exploration of the offshore gas field, the appraisal well and seismic data were limited on the sea, the geology surrounding the gas field area is clear, including the type of structure, and reservoir-cap conditions. Therefore, if inject on land or near shore, relevant exploration needs to be carried out to determine the thickness, occurrence, and distribution range of the high permeability saltwater layer and the cap layer. In consequence, CO2 in the offshore gas fields is more suitable for deep saline water storage mode. According to the method of carbon dioxide capture, the geological storage in the saltwater layer can be divided into primary and secondary capture. Primary capture is the sequestration of carbon dioxide directly blocked by geological traps. Secondary capture means the carbon dioxide is dissolved in salt water and reservoir minerals to produce new minerals and achieve permanent storage of carbon dioxide. Secondary capture is known as mineral carbonization or mineralization and provides a potential method for achieving long-term and permanent storage of CO2. The primary and secondary carbon capture will undergo certain steps as, Primary Capture Treatment : Capture: CO2 emissions are captured from Industrial sources using various technologies such as amine scrubbing, chemical absorption, or other capture methods. Compression and Transportation: The captured CO2 is compressed to increase its density, making it easier to transport. It is then transported via pipelines or other means to the chosen geological storage site, which is often located in deep ground. Injection and Sequestration: The CO2 is injected into the geological trap or formation, where it is intended to be stored securely over long periods. The geological formations act as natural containment structures, preventing the CO2 from escaping back into the atmosphere. Monitoring and Verification: After injection, continuous monitoring and verification are essential to ensure the CO2 remains surely trapped and does not leak back into the atmosphere. Secondary capture Treatment: Pre-treatment: The captured CO2 from primary carbon capture is subjected to the pre-treatment. The pre-treatment unit is comprised of some chemical reactions to remove the impurities. The impurities are collected to the impurity area and the pure Carbon dioxide is taken to the next step. After this pre-treatment, the Carbon dioxide is carried deep into the ocean /Sea. Injection: The purified CO2 is injected into the saline aquifers. The saline aquifers are nothing but solid rocks with millions of pores. According to science in the deep sea, the rock is always pores in nature. So this carbon dioxide fits into the porous of the rock. There are three different types of accommodation of carbon dioxide inside the pores of the rock. If the CO2 settles between the pore spaces is termed residual trapping. If the CO2 dissolves into the other fluids already trapped in the reservoir is termed solution trapping. If the CO2 molecules interact with existing minerals and bind to the rock is termed a Mineral Trapping. How does the CO2 stay underground? A non-porous impermeable cap rock makes it impossible for CO2 to seep upwards, this caprock extends for hundreds of meters permanently sequestering the CO2 below back at the surface our monitoring system fail-safe redundancy and 24-hour surveillance ensures a safe operation for the environment and the community. The above image is the representation of CCUS for both onshore and offshore platforms but the storage is kept deep down the sea. Case study of storage capacity by region The potential for carbon dioxide storage under the sea will depend on various factors such as the geology of the seabed, the presence of suitable geological formations like saline aquifers or depleted oil and gas reservoirs, and the availability of appropriate infrastructure for carbon capture and storage (CCS) projects. The above representation of CO2 storage capacity by region. It will depend upon several factors. One case study I took for the United States. Conservative estimates show that the US has 8.3 trillion metric tons of CO2 storage capacity, that’s enough to store the annual emissions of 35 billion vehicles for the next 50 years.
- HOW MUCH IS A METRIC TON (TONNE)?
It's hard to imagine an invisible gas having much weight, let alone a weighing ton. A metric ton is the unit of mass that scientists use to measure the weight of carbon dioxide. One Metric ton = 1000 kg(or 2204.6 pounds) and is about the same as the weight of about 440 bricks or a great white shark. Imagining carbon emissions One metric ton of carbon dioxide takes up about as much space as a 10-meter cube - a cube whose sides would equal the weight of the length of a telephone pole. In new york city, nearly two metric tons of carbon are emitted every second, mainly by buildings that burn fossil fuels to generate electricity. That's about 150,000 metric tons of carbon per day. In the united states and Canada, each citizen averages a little over 14 metric tons of carbon emissions per year (the weight of about 6,300 bricks). To reach our goal of net zero by 2050, carbon emissions in the atmosphere need to end up at about one metric ton per person ( or approximately 440 bricks). Cutting emissions from 6,300 to 440 bricks per person will require individuals to Eliminate luxuries like airplane travel, plastics, air conditioning, and eating meat. Elect officials to enact sweeping national change, shifting our energy sources from carbon-polluting fossil fuels to renewables like solar and wind. Pressure large businesses to do business in a climate-friendly way. Invest in carbon removal technologies and reforestation to balance the carbon still emitted. Citizens of China emit only one-third as much carbon as Americans, but China leads the world in emissions since China's population is so large. In smaller countries like Mozambique, each citizen already averages less than one metric ton of carbon per year. Technology that removes carbon from the atmosphere has yet to be perfected, so it's impossible to predict precisely how much carbon emissions each individual will actually have to cut to reach net-zero emissions.
- Space Sustainability - Space Debris
Around 5,000 active satellites are currently orbiting the earth and providing tangible social scientific security and economic benefits to billions of individuals all over the globe. This includes enhancing things that we rely upon on a daily basis such as Phones, the Internet, E-portal, Navigation, Weather forecasting, News, Entertainment, etc . Indeed space system applications are now so intimately woven into the fabric of our modern information. However, the ability to continue to provide these important benefits from outer space is now threatened by a number of challenges. The earth's orbital space environment is a finite resource and it's being used by an increasing number of space actors in potentially unsustainable ways. In the early stage of the space age, there are only a few possibilities of a disaster such as space weather and impacts from meteoroids. After the technology emerges to the globe, all countries are involved in space research. This causes the space environment to become much more congested. The number of satellites putting into space is increasing rapidly and there are also tens of thousands of defunct satellites rocket bodies and other fragments that we collectively refer to as "SPACE DEBRIS". According to Google Space Debris is defined as "Space junk, or space debris, is any piece of machinery or debris left by humans in space . It can refer to big objects such as dead satellites that have failed or been left in orbit at the end of their mission". All of this poses an increasingly serious collision hazard to operational satellites and human space flight. There are currently 28,000 pieces of human-generated debris in Earth's orbit layer 10 cm in size each of which could destroy an active satellite in collision. Statistical modeling indicates there are an estimated 7,00,000 pieces of orbital debris between 1 and 10 cm in size that are largely untracked. There are millions of space debris that is less than 1 cm in size. Since some space debris is even less than mm's. It is hard to measure the exact count of space debris that is available. This existing orbital debris is largely concentrated in the same altitudes that are heavily used by satellites and in particular low earth orbit and geostationary earth orbit. A famous scientist Donald J. Kessler in 1978 summarized a phenomenon in which the amount of junk in orbit around Earth reaches a point where it just creates more and more space debris , causing big problems for satellites, astronauts, and mission planners. It is termed as Kessler Syndrome. Kessler also predicts that there would be a critical point where the density of orbital debris would result in random collisions between debris and objects. These random collisions would generate more debris at a rate faster than it is naturally removed from orbit by the earth's atmosphere although this process takes place relatively slowly over decades or centuries we are already seeing it impose a cost on space activities. Satellites operating in congested regions have to manage a growing number of close approach warnings and may need to expand precious fuel to avoid potential collisions with debris. As the amount of orbital debris grows so do the risks of collision making some activities too unsafe risky or unprofitable to continue. In the early days of the space age, the financial and technological barriers to becoming space stars were very high. Only a few countries had the competencies to perform that. But now, the scenario is different. Many more affordable options for launching satellites into space. This is driving innovation of new space technologies and space applications of innovation of new space technologies and space applications at a pace never seen before together these factors have led to an explosion of space activities all around the world. We are also seeing the emergence of space systems with hundreds and even thousands of satellites. Space Debris Comparision from 1957 to 2030 Resolving Space Debris is a complex issue and challenging task that requires international cooperation and the development of advanced technologies. Some strategies have been proposed and are currently being explored to address space debris. They are, Limiting Future Debris - Implementing strict regulations on satellite design and launch procedures to minimize the creation of new debris is crucial. Harpoon, Nets, or Grapple Systems - Concepts involve capturing debris using harpoons, nets, or robotic arms, then deorbiting or safely disposing of them. Laser systems - Ground-based lasers or lasers on spacecraft can be used to change the orbits of small debris, causing them to reenter the earth's atmosphere. Space Traffic Management - Establishing international guidelines and regulations for satellite operators to ensure responsible behavior and coordination in space. Technological Innovation -Developing advanced tracking and monitoring systems to better track the location and movement of space debris. Advancing propulsion technologies that enable more efficient and cost-effective debris removal. Researching new materials for satellites that burn up more completely upon reentry. It's important to note that space debris is a global problem, and addressing it requires international cooperation, long-term commitment, and significant investment in research and development. Additionally, any actions taken to remove space debris must be carefully coordinated to avoid creating more debris in the process.
- Digital Innovations Driving Green Finance: Carbon Trade and Digital Twin
Green finance and digital transformation are now deeply interconnected, enabling businesses to scale sustainability efforts, optimize resource use, and meet environmental, social, and governance (ESG) targets. Technologies such as AI, blockchain, data analytics, IoT, and digital platforms are reshaping the green finance landscape. In this article, I will give proof with some business case study, so that we can relate the practical problems and solutions. Business case study -1: Have you ever wondered if you could trade carbon like equity or a commodity? Yes there is a platform to trade carbon, AirCarbon Exchange is a digital platform focused on carbon credit trading. It provides companies across various industries, such as aviation, logistics, and manufacturing, an opportunity to buy and sell carbon credits to offset their carbon emissions. By leveraging blockchain technology, AirCarbon ensures that every transaction on its platform is transparent, traceable, and tamper-proof. This approach addresses the inefficiencies and opacity that have historically plagued carbon credit markets, making it easier for businesses to engage in carbon offsetting and comply with sustainability goals. So, what could be the outcome of this? In 2022, AirCarbon facilitated the trade of over 10 million carbon credits , which represent approximately 3 million tons of CO2 offset . This is a significant achievement, especially in the context of high-emission sectors like aviation and logistics, which are responsible for substantial carbon emissions. In 2023, the AirCarbon Exchange continued its robust growth, helping to expand the carbon credit trading market with new milestones. In the first half of 2023 alone, the platform reported a 21% increase in trading volumes compared to the same period in 2022, further solidifying its role in scaling carbon offset markets The exchange not only promotes carbon neutrality but also utilizes blockchain technology for improved transparency and reduced transaction costs, contributing to more efficient carbon credit markets. If you clearly look at the business. The goal is to make steel, power, green energy, or any other source. But, the common thing between different businesses is cost and making a sustainable future. Is there a reduction in cost? Previously, the carbon credit trading market relied on intermediaries—such as brokers, auditors, and third-party verifiers—to ensure that carbon credits were legitimate and properly tracked. These intermediaries often added significant transaction fees , increased administrative overhead, and lengthened the process. So, there comes in a blockchain technology. Blockchain technology is known for creating transparent, immutable transaction records. This allows all participants on the platform to view and verify each transaction, making it tamper-proof. The absence of central authorities and intermediaries means that trust and validation are automatically embedded into the system. This technology ensures that transactions are verified on the blockchain with no need for a central authority, cutting out the costs associated with third-party oversight, regulatory approval, and other verification processes traditionally required in carbon credit trading. As a result, transaction costs have been reduced by up to 40% , making the market more efficient and accessible for businesses of all sizes, from large corporations to small and medium enterprises (SMEs) . Benefits of Lower Transaction Costs: With the cost of trading reduced, small and medium enterprises are now better able to participate in the carbon credit market. In the past, these businesses were often excluded from the market due to high entry costs associated with intermediaries. By lowering transaction fees, AirCarbon has created a more competitive marketplace , where carbon credits can be bought and sold at lower prices. This helps increase market participation and reduces the overall cost for companies trying to meet sustainability targets. Lower costs and easier access to carbon credits have helped increase liquidity in the market. This allows for more transactions and a healthier, more active marketplace, which benefits both buyers and sellers. Business case study -2: Digital Twin Technology for ESG Performance Optimization Digital twins are virtual replicas of physical assets that simulate real-time operations, enabling companies to optimize the performance of their physical systems. In one of the company's cases, these digital twins are used to simulate energy consumption, predict maintenance needs, and track carbon emissions, leading to enhanced operational efficiency and better alignment with ESG (Environmental, Social, and Governance) targets. Everyone knows that the CAPEX for Digital Twin is high. Then what will be the outcome? There is a fact that the CAPEX for DT is high but the TOTEX (CAPEX + OPEX) is significantly low when compared with a non-DT Plant / Project. Let's take a look at one business case study. Along with this, there are some benefits, lets look into the benefits part By using digital twin technology, the company was able to simulate and optimize energy usage across its factories. The virtual models helped the company identify inefficiencies and areas where energy consumption could be minimized. This led to an overall 15% reduction in energy consumption across its manufacturing units. This reduction not only lowered operational costs but also helped the company meet its sustainability goals, contributing to a smaller carbon footprint . Optimized energy management was particularly crucial for the company, which has numerous energy-intensive manufacturing processes. 10% Annual Reduction in Carbon Emissions : The ability to continuously track and simulate emission levels enabled the company to adjust energy systems and production processes to reduce carbon emissions by 10% annually . For example, adjustments in heating, cooling, and lighting systems were made based on real-time data from the digital twins, helping to eliminate energy waste. Predictive maintenance is a core advantage of digital twin technology , allowing companies to foresee potential equipment failures before they occur. By applying predictive maintenance, company has been able to reduce maintenance costs by 25% . This has been achieved by using digital twins to monitor and simulate equipment wear and tear. Instead of waiting for a failure to occur, company can now schedule maintenance in advance, avoiding unplanned disruptions and costly emergency repairs. The numbers for energy consumption, carbon emission, and maintenance are great . Then how about the cost? Through energy optimization, emissions reduction, and predictive maintenance, the Company saved an estimated $50 million over two years . These savings were reinvested in further sustainable innovations and operational improvements. Conclusion: Accelerating Green Finance with Digital Transformation Through the strategic use of digital technologies—ranging from AI to blockchain, IoT, and digital twins—companies are not only enhancing their sustainability performance but also reducing operational costs, optimizing investments, and improving transparency in green finance. These digital solutions are not just creating new opportunities for growth in sustainable sectors but also driving long-term value for investors and stakeholders, aligning financial returns with positive environmental impact. As a result, the combined efforts of green finance and digital transformation are accelerating the transition to a more sustainable, carbon-neutral global economy. Key Takeaways: Companies are saving costs through automation and predictive technologies, reducing operational inefficiencies by up to 40% . Digital solutions enhance transparency and trust in green finance, improving investor confidence by 25% . Sustainable investments are growing at a 30% annual rate, outpacing traditional investments by significant margins. By continuing to integrate and scale these digital innovations, businesses can drive substantial progress toward carbon neutrality while delivering financial value to their investors and stakeholders.
- Turnstile Turbine - Paris generates clean energy from Metro Passengers
From the World Economic Forum (WEF) Energy from IBERDROLA converted 6 turnstiles into mini-turbines at Miromesnil Metro station. For 2 days last year, as each passenger passed through the turnstiles, the turbines converted their moment into green energy. 27,000 passengers used the turnstiles. The potential of Turnstile Turbines Technology - If scaled across the entire Metro system, which serves "1.5 Billion passengers annually, this technology could generate 136 MW per year and save 30,000 tonnes of CO2 emissions annually." The aim of this technology was to raise awareness about the energy transition and the challenges we face in getting there. Global renewable energy capacity grew by almost 10% last year, but it needs to increase 3 times faster by 2030 if we are to limit global warming to 1.5 °C or less. The link of the youtube article is as : https://www.youtube.com/watch?v=h-MislTxhKA&t=5s A turnstile wind turbine, also known as a vertical-axis wind turbine (VAWT), is a type of wind energy device that differs in design and operation from the more common horizontal-axis wind turbines (HAWT). While HAWTs have large blades that rotate around a horizontal axis, VAWTs have blades that spin around a vertical axis, much like a turnstile or a merry-go-round. The first ever underground wind turbine that turns commuter's energy into green energy. The system is inspired by automatic watches and real wind turbine mechanisms. The commuter operates the blades as a usual turnstile it drives a series of multiple gears which rotate a flywheel powering a direct current motor. The initial challenge was to transform a very small movement into continuous energy. This challenge becomes the opportunity and the turbine produces more than 2000 watts per day. Turnstile wind turbine generates enough energy to power an entire line and saves more than 30,000 tonnes of CO2 per year . 30,000 tonnes is equal to the following factors, To conclude, the turnstile wind turbine is a unique and innovative technology that harnesses wind or human movement to generate electricity. It's important to note that the energy output of turnstile turbines is relatively modest compared to traditional wind turbines. They are most effective in locations with high foot traffic or consistent wind patterns.
- Offshore Wind Energy - Global market, cost to value, and mid-term prediction
Global opportunities and challenges are manifold, but both are faced with the pressure of time and scale in the fight against climate change. Under scenarios outlined by the world’s leading energy institutions, including the International Agency-Energy (IEA) and the International Renewable Energy Agency (IRENA), achieving carbon neutrality by 2050 – a commitment shared by a majority of countries and conglomerates – will call for rapid and tremendous growth in offshore wind installed capacity. The global market potential for offshore floating wind is significant. Vast wind resources offshore combined with large water depths make floating wind solutions the only realistic option at many locations. According to recent research by McKinsey global installed offshore wind capacity is expected to reach 630 gigawatts (GW) by 2050, up from 40 GW in 2020, and with an upside potential of 1,000 GW in a 1.5° pathway scenario. The global offshore wind market size was valued at $31.8 Billion in 2021 and will reach $56.8 billion by 2026, growing at a compound annual rate (CAGR) of 12.3% from 2021 to 2026. Offshore wind turbines are increasingly being installed and are showing robust growth. Moving from cost to value Offshore wind is a scalable, affordable, and commercially available energy technology. With tremendous capacity to produce power, offshore wind has significant potential to rapidly displace fossil fuels, delivering economic growth and bolstering energy security. After a decade of cost reductions, offshore wind is at an inflection point with a highly competitive levelised cost of electricity (LCOE) that is $3/ MWh below that of coal and $18/ MWh below that of gas. This means that the rate of price reductions that we have seen is likely to slow. Despite this, the cost profile of electricity generated from wind energy versus electricity generation from fossil fuels remains favorable – it has been for some time and will continue to be. Because the offshore wind supply chain is subjected to the fluctuation of commodity prices globally, fluctuations in the cost of wind have to reflect the underlying cost of capital, commodity costs for steel, copper, and other materials, and logistics costs. The past two years have shown the world how volatile the commodity markets are during a global crisis. By embedding market and regulatory imbalances that favor projects with the lowest bids, governments across the world have encouraged a ‘race to the bottom’ on wind pricing. This, combined with inflationary pressures, has exacerbated the squeeze on profitability for the wind industry. Limited profitability has in turn led to underinvestment in manufacturing capacity globally, creating the likelihood of supply chain bottlenecks. To meet the increasing offshore wind ambitions globally, massive investments are needed to build up the offshore wind supply chain and deploy offshore wind projects at the pace and scale needed to achieve the 380 GW of cumulative capacity by 2030 projected under IRENA’s 1.5C scenario. To restore and build a healthy offshore wind industry, governments and industry must ensure a balance between the need for affordable energy, delivery of wider socioeconomic benefits, and a sustainable supply chain. Several countries are trying to capture greater socioeconomic value by including non-price criteria (NPC) in project selection. Mid-Term prediction for offshore wind Reality The sector is grappling with the shift from a handful of single turbine demonstrators and the five wind farms that are operating or under construction today: Hywind Scotland & Kincardine (UK); Windfloat Atlantic (Portugal); Hywind Tampen (Norway); and Goto (Japan). Much applied learning needs to come out of the remainder of this decade, though it is likely to be dwarfed by what happens in the 2030s, when the floating offshore wind is expected to really accelerate. Over the next five years, we expect to see a small number of 100–500 MW projects successfully built. The next phase of installation for floating will shift to France and China, which between them has 300 MW of floating projects in pre-construction and is set for construction later this year or next. China is also active in floating offshore wind, with the Fujian Nanri Island 4 MW demonstrator and the 16.6 MW Nezzy demonstrator both heading into construction shortly, as well as the two 100 MW phases of Power China International Group’s PFS-1 Southeast Wanning project. Depending on completion times, this project is expected to become the world’s largest floating offshore wind farm, demonstrating China’s commitment to floating offshore wind, alongside its unequivocal leadership in fixed offshore wind deployment. With the majority of the global floating wind resource in deeper waters, we can see that confidence in the ability of the wind sector to successfully deploy is prompting governments and leasing bodies to prepare and run auctions for the seabed. In 2022/2023, we have seen progress in the UK with ScotWind, INTOG, and the Celtic Seas leasing rounds, as well as activity in Korea, the US, Spain, Portugal, and Norway. The UK has a set of projects in the pipeline, including the TotalEnergies/Simply Blue Erebus project (96 MW), the EdF/TNB Blyth demonstrator (70 MW), and the CIP/ Hexicon Pentland project (100 MW), highlighting the importance of the UK as a place of learning for the global floating market.
- The Next Source for EV?
The main source of Electric Vehicles is batteries, which provide the necessary energy to power the Vehicle's electric motor. The most common type of battery used is Lithium-ion (Li-ion) batteries, although other types such as solid-state batteries are being researched and developed for future use. Let's have a look at the simple composition of Lithium-ion Batteries. Lithium-ion batteries consist of a cathode (positive electrode), an anode (negative electrode), and an electrolyte. Common materials used as cathodes are lithium cobalt oxide (LiCoO2), lithium iron phosphate (LiFePO4), and nickel manganese cobalt oxide (NMC). The anode is typically made of graphite. The electrolyte is a conductive solution that facilitates the movement of lithium ions between the cathode and anode during charging and discharging. Abundant in Nature? When it comes to abundant in nature, Lithium is not as abundant as elements like silicon or aluminum, it is widespread enough and can be economically extracted from various sources, including lithium-rich brine deposits and lithium -containing minerals. Compared to the other cathode materials such as Cobalt and Nickel. Cobalt is a more limited resource, and a significant portion of global cobalt production comes from a few countries. The industry is actively exploring ways to reduce or eliminate cobalt in battery formulations due to ethical and sustainability concerns associated with some cobalt mining practices. Nickel is more abundant than cobalt, and there are efforts to increase the nickel content in batteries, as it allows for higher energy density . However, like cobalt, nickel extraction and processing raise environmental and ethical concerns, leading to increased attention to responsible sourcing. A seemingly simple shift in lithium-ion battery manufacturing could pay big dividends, improving electric vehicles (EV) ability to store more energy per charge and to withstand more charging cycles, according to new research led by the Department of Energy's Pacific Northwest National Laboratory. The nickel-rich battery vision Cathodes for conventional EV batteries use a cocktail of metal oxides—lithium nickel manganese cobalt oxides (LiNi1/3Mn1/3Co1/3O2), abbreviated NMC. When more nickel is incorporated into a cathode, it greatly increases the battery's ability to store energy, and thus, the range of the EV. As a result, nickel-rich NMC (such as NMC811, where the "8" denotes 80% nickel, the first "1" denotes 10% cobalt, and the second "1" denotes 10% Manganese(8:1:1)) is of great interest and importance. The most significant feature of the NMC 811 battery is its high energy density and reduced cost of EV Batteries. This comes from the Nickel in the cathode which can increase the material activity and thus the energy density. Additionally, the high Nickel content is important for increasing the capacity. The cobalt is also an active component that stabilizes the laminar structure of the material, thereby increasing the discharge capacity of the material. The manganese component, which plays a supporting role in the electrodes provides stability during charging and discharging. However, high-nickel NMC cathodes formed using the standard method are agglomerated into polycrystal structures that are rough and lumpy. This meatball-like texture has its advantages over regular NMC. For NMC811 and beyond, though, the bulbous polycrystal fissures are prone to splitting apart, causing material failure. This renders batteries made using these nickel-rich cathodes susceptible to cracking, they also begin to produce gases and decay faster than cathodes with less nickel. Challenges of synthesizing single-crystal NMC811 One strategy to fix this problem - convert that lumpy, polycrystal NMC into a smooth, single-crystal form by eliminating the problematic boundaries between the crystals—but this conversion is easier said than done. In laboratories, single crystals are grown in environments such as molten salts or hydrothermal reactions that produce smooth crystal surfaces. However, these environments are not practical for real-world cathode manufacturing, where lower-cost, solid-state methods are preferred. In these more typical solid-state approaches, an NMC cathode is prepared by mixing a metal hydroxide precursor with a lithium salt, directly mixing and heating those hydroxides, and producing the agglomerated (lumpily clustered) polycrystal NMC. Using a multiple-step heating process results in micron-sized crystals, but they are still agglomerated, so the undesirable side effects persist. Solution Employ advanced synthesis techniques to produce high-quality single crystal or polycrystalline NMC 811 cathode material. Improve the electrochemical performance of NMC 811 to enhance battery efficiency and longevity. Develop cost-effective methods for synthesizing NMC 811 without compromising performance. Facilitate the large-scale production of NMC 811 for commercial applications. Create a resilient and sustainable supply chain for the raw materials used in NMC 811 production. In conclusion, the development and widespread adoption of electric vehicles (EVs) heavily rely on advancements in battery technology, with Lithium-ion (Li-ion) batteries being the primary energy source for EVs. Understanding the composition of Li-ion batteries, particularly the cathode materials such as lithium cobalt oxide (LiCoO2), lithium iron phosphate (LiFePO4), and nickel manganese cobalt oxide (NMC), is crucial for enhancing their performance. While lithium, a key component, is not as abundant as some other elements, its extraction remains economically viable from various sources. However, the industry faces challenges associated with environmental and ethical concerns related to the extraction of materials like cobalt and nickel. The research spotlight has shifted toward nickel-rich NMC cathodes, such as NMC811, which boasts an 80% nickel composition. This shift aims to improve energy density, extend the range of EVs, and reduce costs. The high nickel content enhances material activity and increases capacity, while cobalt stabilizes the material's structure. However, challenges arise in synthesizing single-crystal NMC811 due to the current polycrystalline structures being prone to splitting and material failure. Addressing these challenges requires advanced synthesis techniques to produce high-quality single-crystal or polycrystalline NMC811 cathode material. Additionally, efforts should focus on improving electrochemical performance, developing cost-effective synthesis methods, and facilitating large-scale production for commercial applications. It is equally important to establish a resilient and sustainable supply chain for raw materials, considering the environmental and ethical concerns associated with their extraction. By tackling these aspects, the electric vehicle industry can enhance the efficiency, longevity, and overall sustainability of Li-ion batteries, contributing to the broader goal of transitioning to cleaner and more sustainable transportation solutions
- Sustainable Aviation Fuel
SAF uses feedstocks such as cooking oil to reduce emissions by up to 80 percent. One advantage of SAF is that it can be blended with traditional jet fuel and used with existing infrastructure, engines, and planes. SAF gives an impressive reduction of up to 80% in carbon emissions over the fuel lifecycle compared to the traditional jet fuel it replaces, depending on the sustainable feedstock used, production method, and the supply chain to the airport. According to the International Civil Aviation Organization (ICAO), over 360,000 commercial flights have used SAF at 46 different airports largely concentrated in the United States and Europe. Worldwide, aviation accounts for 2% of all human-caused carbon dioxide (CO2) emissions and 12% of all transportation CO2 emissions. ICAO's Carbon Offsetting and Reduction Scheme for International Aviation (CORSIA) caps net CO2 aviation emissions at 2020 levels through 2035. The international aviation industry has set an aspirational goal to reach net zero carbon by 2050 . SAF presents the best near-term opportunity to meet these goals. The Sustainable Aviation Fuel Grand Challenge, announced in 2021, brings together multiple federal agencies to expand domestic consumption to 3 billion gallons in 2030 and 35 billion gallons in 2050 while achieving at least a 50% reduction in lifecycle greenhouse gas emissions. SAF can also be produced using renewable feedstocks such as agricultural or forestry waste. When produced from renewable feedstocks SAF only emits the same amount of carbon to the atmosphere as was previously absorbed by its feedstock, thereby closing the carbon loop. What are the benefits of SAF compared to fossil jet fuel? Based on our Life Cycle Analysis, a specific batch of SAF can reduce emissions by 75% or more, compared to fossil jet fuel over its entire life span. This includes production, distribution, transportation, and combustion. It can also reduce other harmful emissions like particulates and sulfur by 90% and 100% respectively. These reductions are critical ways to reduce the impact of climate change on our planet. International Air Transport Association (IATA) estimates that SAF could contribute around 65% of the reduction in emissions needed by aviation to reach net zero in 2050. This will require a massive increase in production. The largest acceleration is expected in the 2030s as policy support becomes global, SAF becomes competitive with fossil kerosene and credible offsets become scarcer. Evolution of SAF : 2008: The first test flight with bio jet fuel was performed by Virgin Atlantic. 2011–2015: 22 airlines performed over 2,500 commercial passenger flights with blends of up to 50% bio jet fuel from feedstock including used cooking oil, jatropha, camelina, and algae. January 2016: Regular sustainable fuel supply through the common hydrant system started at Oslo Airport. Alternative fuel producer Neste and supplier SkyNRG as well as Air BP involved. March 2016: United became the first airline to introduce SAF into normal business operations by commencing daily flights from Los Angeles Airport (LAX), supplied by AltAir. June 2017: At the 73rd IATA AGM in Cancun, IATA members unanimously agreed on a resolution on the deployment of SAF, including calling for constructive government policies, and committing to only use fuels that conserve ecological balance and avoid depletion of natural resources. November 2019: Commercial SAF flights exceed 250,000 and more than 45 airlines gain experience using SAF. June 2020: Two new technical SAF certifications are approved by ASTM increasing the approved technical pathways for SAF production to seven October 2021: The 77th IATA AGM in Boston approved a resolution for the global air transport industry to achieve net-zero carbon emissions by 2050. This commitment aligns with the Paris Agreement goal of limiting global warming well below 2°C. A potential scenario is that 65% of this will be abated through SAF. October 2022: adoption of a Long Term Aspirational Goal (LTAG) to achieve net zero CO2 emissions by 2050 at the 41st ICAO Assembly. In 2022, SAF production tripled to 300 million liters from 100 million liters in 2021. In June 2023, IATA released a series of strategic roadmaps to showcase the critical steps to reach net zero by 2050, including SAF. In October 2023, the EU adopted ReFuelEU Aviation which completed the ‘Fit for 55’ legislation. Aviation fuel suppliers will have to blend increasing amounts of SAF with kerosene, starting with a 2% minimum blend in 2025, and rising to 70% in 2050. In 2023, SAF production tripled to 600 million liters from 300 million liters in 2022, representing 0.2% of global jet fuel use.
- The Evolution : The First Electric Vehicle
As we're all aware, electric vehicles (EVs) have become a prevalent mode of transportation in our modern era. Have you ever paused to consider what the very first electric vehicle was like? Imagining its design and understanding how it worked can provide fascinating insights into the evolution of this revolutionary technology. The concept of electric vehicles dates back further than many might think. The first known electric vehicle was invented in the early 19th century. It was a modest carriage designed by Robert Anderson, a Scottish inventor , in the 1830s. However, it wasn't until the late 1800s and early 1900s that electric vehicles began to gain traction. The First Ever Electric Car-Invented in 1832 It was designed more like a carriage than a car due to the evolution from the horse and carriage which was their usual mode of transport. The carriage was powered by non-rechargeable power cells so when the batteries ran out, they would need to be replaced. Following this invention, in 1835 Thomas Davenport launched a small vehicle powered by the first American DC electric motor. Evolution of Rechargeable Batteries - As the popularity of electric-powered transport grew, 1859 brought the invention of rechargeable batteries ; this was the only major development since the launch of Thomas Davenport’s small electric car. Two major improvements then occurred in 1884. First, Thomas Parker built the first electric car production line in London and secondly, William Morrison introduced a simple electric wagon to the USA. These innovations led to electric vehicles becoming very popular, and soon became the preferred method of transport as they didn’t produce emissions, were very quiet, and were easy to drive. Ferdinand Porsche , the founder of Porsche, developed an electric car called the P1 . This model, created in 1898, was one of the first steps that helped create the luxury car brand that is seen today. So, if you see a Porsche Taycan roaming the streets, they carry a lot more history behind them than you may think! By the start of the 1900s one-third of the vehicles on US roads were powered by electricity. Thomas Edison, an American inventor and businessman believed this mode of transport was superior therefore, he decided to study better ways to build electric batteries. The flair for electric vehicles lasted until 1908 when Henry Ford launched the world’s first affordable motor car, the Model T . This car was an overwhelming success and became widely available. Consequently, the electric car developments stopped due to being significantly more expensive. A gap in the market for alternatively fueled cars appeared in the 1960s and 70s since petrol prices went soaring. However, the government’s unwillingness to support the technology, due to oil continuing to be able to generate masses of revenue, meant that the electric car industry never fully regained its popularity. A British Chemist named M. Stanley Whittingham invented the world’s first rechargeable lithium-ion batteries in 1973 . These batteries are still used in today’s mobile phones, laptops, and electric cars. This encouraged electric cars to return, and development re-started. However, then came the fall of petrol prices, affecting the interest on EVs and causing it to spiral yet again. 1997 brought the unveiling of the hybrid Toyota Prius , the world’s first mass-produced hybrid vehicle. Toyota helped pave the way for other automotive companies to delve into the electric car scene. Tesla unveiled the Roadster in 2008 showing off their front-line technology and powertrain. Nissan followed shortly after and launched the Nissan Leaf, a small 5-door fully electric hatchback. The design of these early electric vehicles was quite different from the sleek and efficient models we see today. In terms of how they worked, early electric vehicles utilized lead-acid batteries to power their electric motors. The batteries were heavy and had limited range compared to today's lithium-ion batteries. Despite these limitations, they were favored for their quiet operation and lack of noxious fumes. Comparison of the first EV and today's EV: The true turning point for electric vehicles came with innovations in battery technology and the rise of environmental awareness in the late 20th century. This led to the development of more efficient and powerful electric vehicles, culminating in the sophisticated EVs we have today. So, the next time you plug in your electric vehicle, take a moment to appreciate the journey from Robert Anderson's humble carriage to the cutting-edge EVs of the 21st century.
- The Evolution: The First Offshore Platform in the World
The first offshore drilling rig is generally considered to be the "Caledon" or "Smith #1" platform , which was built by the California Company and was located off the coast of Summerland, California, USA. photo by G.H. Eldridge courtesy National Oceanic & Atmospheric Administration Have you ever wondered how the first offshore rig design will be? The following design was drawn and published on May 4, 1869. Offshore drilling began in 1897, just 38 years after Col. Edwin Drake drilled the first well in 1859. H.L. Williams is credited with drilling a well off a wooden pier in the Santa Barbara Channel in California. He used the pier to support a land rig next to an existing field. Five years later, there were 150 “offshore” wells in the area. By 1921, steel piers were being used in Rincon and Elwood (California) to support land-type drilling rigs. In 1932, a steel-pier island (60 × 90 ft with a 25-ft air gap) was built ½ mile offshore by a small oil company, Indian Petroleum Corp., to support another onshore-type rig. Although the wells were disappointing and the island was destroyed in 1940 by a storm, it was the forerunner of the steel-jacketed platforms of today. Location: Summerland, California, USA Year Established: 1896 Type: Wooden structure, resembling a pier with drilling equipment Innovation: The Caledon platform was a pioneering effort in offshore drilling. It utilized a wooden structure, resembling a pier, extending from the shoreline into the ocean. This structure supported drilling equipment, allowing drilling operations to take place over the water. Depth: It was situated in about 9 meters (30 feet) of water . If you want to explore more on this particular platform? Structure and Design: Resembling a substantial pier extending from the shoreline into the waters, the Caledon platform was constructed entirely from wood. Its design, though simple by modern standards, was an engineering marvel for its era. Equipped with essential drilling apparatus, the platform facilitated the exploration and extraction of oil from beneath the ocean's surface . Historical Significance: The establishment of the Caledon platform marked a pivotal moment in the quest for offshore oil reserves. It served as the vanguard, demonstrating the feasibility and potential of drilling operations over open water. The success of this platform paved the way for further advancements in offshore drilling technology and practices, shaping the landscape of the global oil and gas industry. Following the success of the Caledon platform, the industry began to develop more sophisticated offshore drilling technologies and structures, such as steel platforms and floating rigs. These developments laid the foundation for the extensive offshore drilling operations seen today in various parts of the world, from the Gulf of Mexico to the North Sea and beyond. The Caledon (Smith #1) platform remains an important landmark in the history of offshore drilling, symbolizing the beginning of a new era in oil exploration and production.
- Why do Business leaders look to Quantum Computing ?
Quantum Computing, a field once limited to academic research is rapidly gaining attention from business leaders across various industries. As companies strive for innovation, efficiency, and competitive advantage in the digital age, quantum computing presents transformative opportunities that could reshape everything from logistics to pharmaceuticals. But Why are so many business leaders turning their focus toward this technology? The answer lies in quantum computing's potential to solve problems that were previously considered unsolvable, which now offers a leap forward in computational power and problem-solving capabilities. Business leaders are visionary, problem solvers and they can easily look to the future. So Quantum computing can be the core pillar for future-proofing business. However, quantum computing is still in its early stages, with large-scale, commercially viable quantum systems yet to be fully realized. However, the pace of advancement is accelerating, with companies like IBM, Google, and Microsoft making significant strides in developing quantum technology. By investing in quantum research today, business leaders are positioning their organizations for a future where quantum computing could become a competitive necessity. The global quantum computing market, valued at $472 million in 2021 , is projected to grow to $4.8 billion by 2029 , reflecting a compound annual growth rate (CAGR) of 31.2% . Those who embrace quantum technology early are likely to enjoy first-mover advantages, accessing new solutions, optimizing operations, and potentially unlocking entirely new business models. Forward-thinking companies across industries are forming partnerships with quantum computing firms and dedicating resources to quantum research to stay ahead of the curve. The other reason is the rapid growth of Artificial Intelligence and Machine Learning technologies. We know that AI & ML are the key drivers of innovation in modern business, powering everything from personal marketing to predictive analytics. For instance, machine learning could be trained exponentially faster using quantum computing, resulting in more accurate predictions and faster decision-making. Quantum machine learning (QML) could reduce the time it takes to train models exponentially. For instance, in 2021, Cambridge Quantum demonstrated how quantum computing could enhance natural language processing, offering more accurate insights in fields like customer service and fraud detection. Business leaders who rely on AI for critical functions, such as customer insights, fraud detection, or predictive maintenance, are eager to tap into quantum advantage. Beyond its practical applications, quantum computing also opens up a realm of possibilities for disruption innovation. By solving complex problems that were previously unsolvable, quantum technology could drive entirely breakthroughs in areas like material science, drug discovery, and climate modeling. For business leaders, the chance to pioneer these breakthroughs presents an enticing opportunity for growth and market leadership. For example, in materials science, the quantum computing cloud enables the discovery of new materials with specific properties, leading to advancements in industries like energy storage, aerospace, and manufacturing. Similarly, in healthcare, quantum simulations could allow for personalized medicine by accurately modeling how different treatments interact with an individual's unique genetic makeup. The race to quantum computing is not just about technology, it's about survival in a future where data, speed, and optimization define success. Business leaders who understand this potential are investing in the future of quantum computing, recognizing that its capabilities could disrupt entire industries and create new opportunities. While the technology is still in development, it has the potential to revolutionize various fields. By positioning themselves early, companies can prepare for the quantum revolution and lead the charge into a new era of innovation and technology assessment. The question is no longer if quantum computing will change the business landscape, but when and the smart leaders are preparing for that moment today.